Understanding MW vs MWh: Power and Energy Explained
.png)
How Do You Measure Energy?
The energy transition is bringing new voices to the energy sector. From social media debates about nuclear versus renewables, to journalists covering industry news, policy announcements, and the new records being broken almost every day.
It's great that more people are engaged in the energy transition, but we often see confusion about the units we use in the power industry: mixing up MW and MWh, or incorrectly writing MW/h for MWh.
And even when you get your MW and MWh straight, it can be difficult to interpret these quantities correctly to compare different technologies and different energy sources. So in this article we’re going to dig into how to measure energy in the power system.
On megawatts and megawatt-hours
We use different units of measure in different domains. Engineers working on power systems need different measurements than nutritionists studying food energy or physicists analysing particle interactions.
Even though the calories in a muffin, the megajoules in a litre of petrol, and the kilowatt-hours of energy produced by some solar panels are all in some sense directly equivalent, the different units we use are useful for their specific contexts.
In power systems, megawatts (MW) measure instantaneous power - the rate at which energy is being generated, transmitted, or consumed at any moment. When measuring energy delivered or consumed over a period of time, we use megawatt-hours (MWh).
The difference between power and energy becomes clearer with an analogy: think of a water hose filling a swimming pool. The flow rate of the hose - say, 100 liters per minute - is like power (MW). It tells you how quickly water is flowing. The total water in the pool is like energy (MWh) - it's what accumulates over time.
If your 100-liter-per-minute hose runs for an hour, you'll have added 6,000 liters to the pool. Similarly, a 100 MW power plant running for one hour delivers 100 MWh of energy.
One common error we sometimes see is people writing "MW/h" when meaning MWh. MW/h would mean megawatts per hour - a rate of change of power, like saying "the power plant's output is increasing by 5 MW/h”.
Just as we wouldn't say "liters per minute per hour" to measure delivered water, we don't say "MW/h" to measure delivered energy.
While we’re being pedantic about our MWs and MWhs [sic] also remember that we shouldn’t ever add a plural ‘s’ because they are part of the International System of Units and SI units and their derivatives don't take a plural 's'.
Electricity Meters
While the focus of this article is understanding how to use the key units of measure in the power system, its worth a brief aside on how these are technically measured.
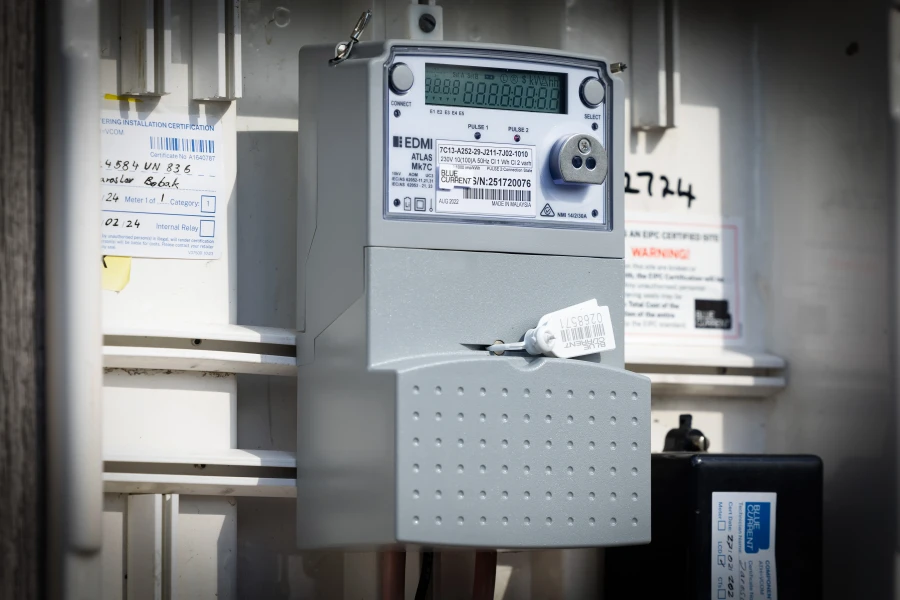
An electricity meter takes measurement using voltage and current transformers: voltage is stepped down to a measurable level, while current is measured when the main power line, or lines for three-phase power, pass through a current transformer (CT). The meter uses these measurements to calculate watts and watt-hours, which is explained further at the bottom of this article.
Are all megawatts created equal?
Are all megawatts equivalent in energy terms? How comparable is 100MW of solar, wind, gas, or battery capacity? The nameplate capacity of a power plant or storage system in megawatts doesn't necessarily predict its energy production: a 1 MW system doesn't necessarily produce 1 MWh of energy every hour.
Capacity Factors
We sometimes talk about this using the concept of a capacity factor - the ratio of actual to theoretical maximum production over time.
For example, we might say that solar PV technology has an average capacity factor of 15% because its actual production over, say, a year, is only 15% of its theoretical maximum due to weather dependence.
But different technologies, different system designs, different markets, and different geographic locations (with different levels of solar and wind resource) can have very, very different capacity factors.
In fact, the variance in capacity factors for different systems within some class of technology, like solar or gas, can be greater than the variance between different classes of technology, so using average capacity factors when debating different energy technology (like saying solar ‘only’ has a capacity factor of 15%) is not very useful.
Did you know a solar system in South Australia will have about twice the annual energy production of an identical system in Saxony in Germany.
And even two systems with the same power rating in the same location can produce different amounts of energy due to having different system designs -- a tracking solar system that follows the sun produces much more energy than a fixed system with sub-optimal orientation and tilt, and oversizing panel capacity relative to inverter capacity can also significantly change the production profile and resulting capacity factor.
Or take a thermal generator like a gas power plant or a nuclear power plant. While we generally consider thermal generators to be schedulable or dispatchable because output doesn't vary based on weather, individual systems never have a capacity factor even close to 100%.
These systems face operational limits from scheduled maintenance, faults (which can be related to weather), and fuel supply issues.
Additionally, all generators will reduce or curtail their output during periods of low or negative wholesale prices when there's excess generation, and can be forced to curtail their output by electricity system operators due to transmission or distribution constraints.
System design can also have a big impact on thermal generation, for example a 'peaking gas plant' may only have enough fuel and will only be designed to run for short periods of time during system stress events or when power prices are very high.
Energy Storage Duration
Battery and pumped-hydro energy storage systems add another dimension. While rated in megawatts (power), their storage capacity in megawatt-hours (duration) determines how long they can operate and this can vary considerably.
An uninterruptible power supply system for a data center might only be able to operate for a few minutes while the backup generators for the site start-up, while on the other hand a large utility-scale pumped hydro system could store days or even weeks worth of energy.
Battery energy storage systems typically store between half an hour and four hours of energy. When thinking about energy storage duration, it's important to understand that this is just the time period over which the storage system can deliver energy at its full power rating. (Check out our blog on battery capacity here).
Consider a two-hour and four-hour battery with the same storage capacity in MWh, say 8 MWh. The four-hour battery will have a power rating of 2 MW and the 2-hour battery will have a power rating of 4 MW.
Both can deliver energy for two hours, but the four hour battery will only be able to discharge half its energy storage capacity in that time.
And both can also deliver energy for four hours, but the two-hour battery will only be using half of its power capacity over that time (it could discharge faster if needed).
Emerging battery technology targeting very long durations, like iron-air batteries aiming for 100-hours of duration, implies battery systems with much lower power capacity (MW) relative to their energy capacity (MWh), and the main feature we are looking for with "long duration" is very low energy storage costs ($/MWh), rather than “long duration” being a technology characteristic in itself.
Are all megawatt-hours created equal?
Similarly not all megawatt-hours are equivalent. This is because of the difference between primary energy and useful energy.
Primary energy refers to the underlying energy content of fuels like coal or gas. Useful energy is what actually performs the intended service - like heating, cooling, illumination, or motion. Different technologies convert primary to useful energy with different efficiencies.
Heating example
Home heating provides a good example of efficiency differences.
A gas boiler converts gas to heat through combustion at about 85% efficiency (if we were trying to produce electricity from gas rather than heat, the efficiency would be much lower).
So for 10 kWh of useful heat energy output, the gas boiler needs 11.8 kWh of primary energy input.
A heat pump works differently - it moves heat rather than generating it. This allows it to deliver 3-4 units of heat energy for every unit of electrical energy consumed (known as the Coefficient of Performance or COP). For 10 kWh of heat output, a heat pump with a COP of 3 needs only 3.3 kWh of electrical input.

So in this case 11.8kWh of gas energy is equivalent to 3.3 kWh of electrical energy.
Cooling example
Here’s another interesting example. Consider an air conditioner powered by two different electricity sources, coal and solar, and let's say we need 3 kWh of electrical energy supplied to our air conditioner to keep our room cool for an hour.
With coal-fired power supply: The coal plant operates at 35% efficiency and there are 8% line losses in transmission and distribution. To deliver 3 kWh to the customer, we first need to generate 3.26 kWh at the power plant (accounting for line losses), which requires 9.3 kWh of primary energy from coal (accounting for plant efficiency).
With rooftop solar power supply: A solar inverter is 97% efficient. To deliver 3 kWh of AC electricity requires 3.1 kWh of DC energy from the panels. The electricity is generated and used in the same location, avoiding transmission and distribution losses (and the associated infrastructure costs).
The concept of primary energy becomes less meaningful with solar power. While we can measure the solar radiation falling on the panels (around 1000 watts per square meter at noon on a clear day), this is a free and functionally unlimited resource rather than a consumable fuel. Solar panel efficiency (around 20%) affects how much panel area we need, but does not represent a loss of purchased or finite primary energy supply.
Let’s get even more technical
Before we wrap, let's dig into energy units a little deeper (or feel free to skip this section if understanding the difference between megawatts and megawatt-hours is enough for you).
Megavolt-ampres
While we've been talking about MW, you might also hear energy professionals talking about MVA, or megavolt-amperes. If you are a commercial or industrial energy user you might even see lines on your bill referring to kVA or MVA.
Here is the difference: megawatts (MW) measure real power and megavolt-amperes (MVA) measure apparent power.
Real power is the power that gets converted into useful work: illumination, cooling, movement, etc. The relationship between MW and MVA is called power factor - a measure of how effectively power is being delivered.
For a given MW (real) load we will need more MVA (apparent) of generation, transmission and distribution capacity to serve that load. The closer these numbers are, and the closer the power factor is to unity, the more efficient the power system.
Power factor
Power factor is a ratio measuring how effectively an AC system delivers real power. In AC circuits, voltage and current can be out of phase, leading to apparent power (MVA) being higher than real power (MW). A power factor of 0.9 is typical in power systems, meaning that 1 MVA of apparent power delivers 0.9 MW of real power.
Low power factors indicate inefficient power delivery and can result from certain types of electrical loads like motors or transformers operating below their rated capacity.
Calculation
Here’s how we convert from MVA to MW. For three-phase AC (Alternating Current) power systems, which is standard for electricity networks, the relationship is:
Power (MW) = Voltage (kV) × Current (A) × √3 × power factor × 10^-3
The √3 factor appears because power is delivered through three conductors (wires) carrying alternating current, with voltages phase-shifted by 120 degrees. This three-phase arrangement is more efficient than single-phase for power transmission. The √3 multiplier accounts for the relationship between the lines (conductors) in this three-phase configuration.
Transmission and distribution example
Transmission and distribution lines are typically classified by their voltage level (kV) — high-voltage, medium voltage and low voltage — but their power carrying capacity can still be calculated in MW using the equation above.
Let's look at a couple of real examples.
Low Voltage Example

At the local level, a distribution transformer might step down voltage to 230V (0.23kV) to serve homes.
A typical residential feeder operating at this voltage with a 400A capacity and 0.9 power factor can deliver 143 kW of power. This capacity might serve, say, 50 homes, assuming typical residential demand patterns where not all homes peak at once.
0.23 kV × 400A × √3 × 0.9 × 10^-3 = 0.143 MW (143 kW)
High Voltage Example
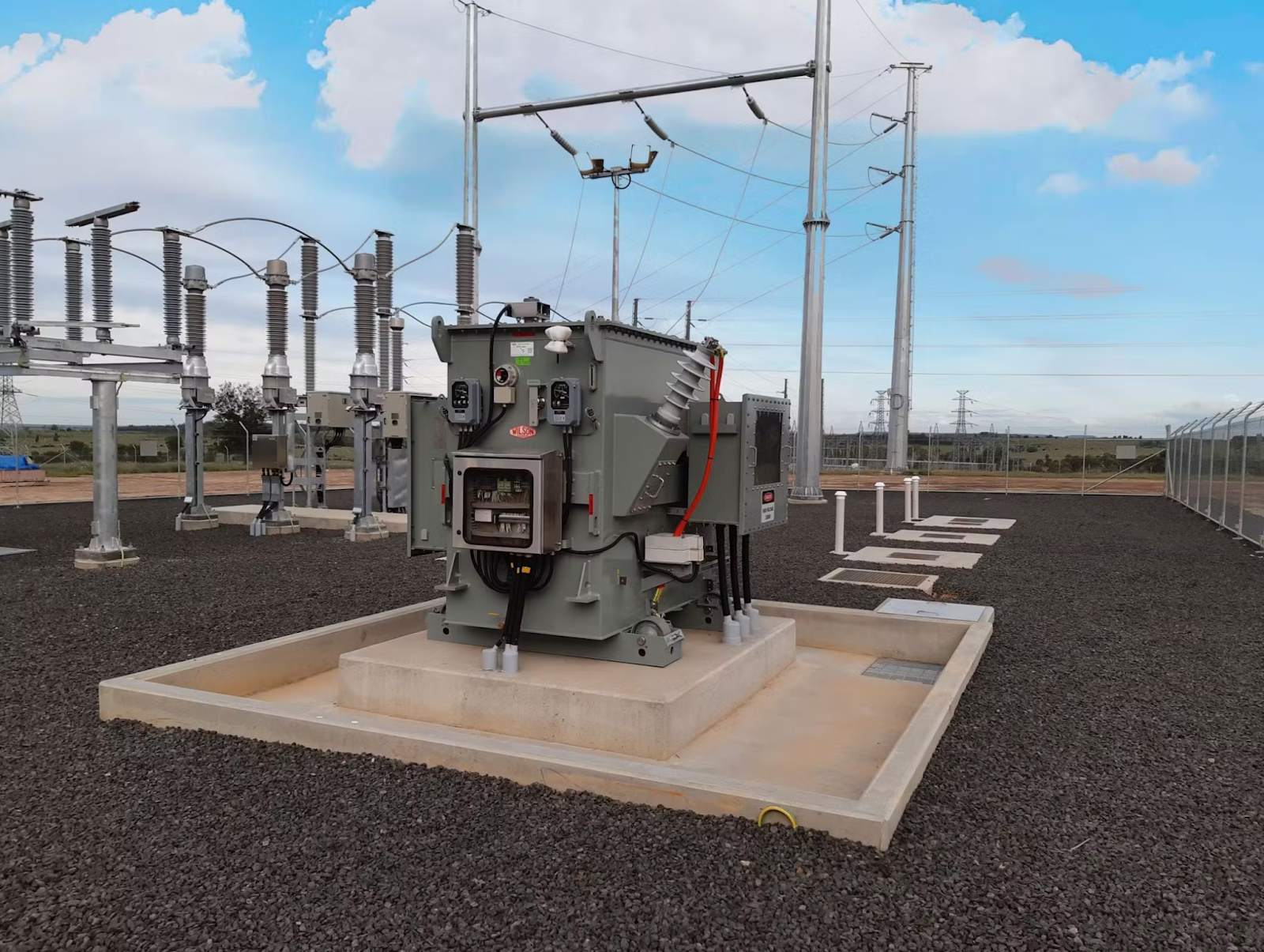
In contrast, a high voltage transmission line operating at, say, 220kV with a 1,000A capacity and 0.95 power factor can deliver 362 MW of power. This transmission capacity is typical of lines connecting power plants to the grid.
220 kV × 1,000A × √3 × 0.95 × 10^-3 = 362 MW
Converting from other energy units
Beyond electrical systems, we can encounter many other energy units like joules, calories, and BTUs. A joule is a very small unit - the energy needed to lift a small apple one meter against Earth's gravity.
One kilowatt-hour equals 3.6 megajoules, providing a bridge between electrical and mechanical/chemical energy measurements.
Here are common conversion factors across different domains:
Power Unit = Equivalent in kW
1 MW = 1,000 kW
1 MVA = 900 kW (at power factor 0.9)
1 horsepower = 0.746 kW
1 BTU/hour = 0.293 kW
Energy Unit = Equivalent in kWh
1 MWh = 1,000 kWh
1 BTU = 0.000293071 kWh
1 Calorie = 0.001163 kWh
1 Joule = 0.000000278 kWh
1 Barrel of Oil = 1,700 kWh
Wrapping Up
Understanding energy units and their relationships helps us compare different energy systems and technologies. Converting between units allows us to analyze energy use across different applications - from power generation to heating and transportation. The relationships between power and energy, and between primary and useful energy, are particularly important for evaluating energy system performance.